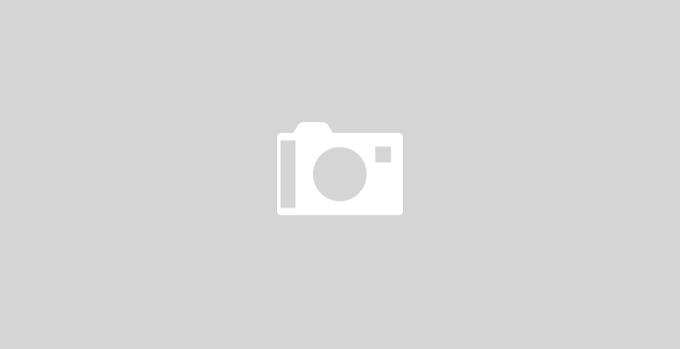
The effect of whitespace and aspect ratio on wirelength and timing
Whitespaces (empty space) are inserted in layouts in order to increase the routing resources of the chip. Have you ever…
Whitespaces (empty space) are inserted in layouts in order to increase the routing resources of the chip. Have you ever…
Sometimes the delay through a component is dependent upon the values on signals. This is because different paths in the…
Because of shrinking feature sizes and the decreasing faithfulness of the manufacturing process to design features, pro…
Register retiming is a sequential optimization technique that moves registers through the combinational logic gates of …
While designing systems with DVFS techniques, we need to look at the impact of temperature inversion on the performance…
Due to a miscommunication during design, you thought your circuit was supposed to have a supply voltage of 2.1 volts (t…
Clock latency means, the number of clock pulses required by the ckt to give out the first output. Generally we will obs…
In the simplest form: FF1 - combo - FF2 ( this is how things look physically for our consideration) Tmin = Tclk2Q (F…
Check critical path and optimize it. Add more timing constraints (over constrain). pipeline the architecture to the max…
An application-specific integrated circuit or ASIC comprises an integrated circuit (IC) with functionality customized f…